<b>Fennel</b>, K., S. R. Alin, L. Barbero, W. Evans, T. Bourgeois, S. R. Cooley, J. Dunne, R. A. Feely, J. M. Hernandez-Ayon, C. Hu, X. Hu, S. E. Lohrenz, F. Muller-Karger, R. G. Najjar, L. Robbins, J. Russell, E. H. Shadwick, S. Siedlecki, N. Steiner, D. Turk, P. Vlahos, and Z. A. Wang, 2018: Chapter 16: Coastal ocean and continental shelves. In Second State of the Carbon Cycle Report (SOCCR2): A Sustained Assessment Report [Cavallaro, N., G. Shrestha, R. Birdsey, M. A. Mayes, R. G. Najjar, S. C. Reed, P. Romero-Lankao, and Z. Zhu (eds.)]. U.S. Global Change Research Program, Washington, DC, USA, pp. 649-688, https://doi.org/10.7930/ SOCCR2.2018.Ch16.
Coastal Ocean and Continental Shelves
16.4.1 Trends in Coastal Carbon Fluxes
Important questions with respect to coastal carbon fluxes include:
What is the anthropogenic component of the CO2 sink?
How will the coastal ocean change as a CO2 sink?
How will changing climate and other forcings affect the total and anthropogenic flux proportions?
As stated in Section 16.2, when considering the ocean’s role in sequestering anthropogenic carbon, the relevant component is anthropogenic flux, not the total uptake flux. Neither quantifying the anthropogenic carbon flux component nor predicting its future trend is straightforward. Here the likely trends in total carbon fluxes are described; by definition, changes in total carbon fluxes imply changes in anthropogenic fluxes as well.
A direct effect of increasing atmospheric CO2 will be an increase in net uptake by the coastal ocean. In addition to rising atmospheric CO2 levels, changes in climate forcings (i.e., surface heat fluxes, winds, and freshwater input) may affect carbon fluxes in North American coastal waters. Ocean warming reduces the solubility of gases and thus directly affects gas concentrations near the surface; this likely will decrease the net air-sea flux of CO2 by reducing the undersaturation of CO2 (see Cahill et al., 2016, for the North American Atlantic Coast). Surface temperature increases also strengthen vertical stratification and thus impede vertical mixing, effects which will affect upward diffusion of nutrients and DIC. Enhanced stratification, therefore, could lead to decreases in both biologically driven carbon uptake and CO2 outgassing. However, model projections for the northern GMx show that the direct effect of increasing atmospheric CO2 overwhelms the other more secondary effects (Laurent et al., 2018). Furthermore, temperature trends in coastal waters around North America show complex patterns with some regions having cooled from 1982 to 1997 followed by warming from 1997 to 2013 (e.g., the MAB), some regions having warmed from 1982 to 1997 followed by cooling from 1997 to 2013 (e.g., the SAB and Gulf of Alaska), and other regions showing no consistent warming from 1982 to 2013 (e.g., the NAA; Liao et al., 2015). Temperature anomalies from a time series in the central California Current System show warm surface waters for the decade prior to 1997 followed by a prolonged cooler period until the strong surface warming associated with a marine heatwave and the 2015 to 2016 El Niño interrupted the cool anomalies (Chavez et al., 2017). However, deeper waters in the California Undercurrent have shown a multidecadal trend (1980 to 2012) toward warmer, saltier, lower-oxygen, and higher-CO2 waters at a depth associated with increased northward transport of Pacific equatorial waters (Meinvielle and Johnson 2013).
Some studies suggest that trends in the air-sea pCO2 gradient (ΔpCO2) are indicative of a strengthening or weakening of the net CO2 uptake by shelf systems, where an increasing ΔpCO2, implying that ocean pCO2 rises more slowly than atmospheric pCO2, corresponds to increased net uptake and cross-shelf export (Laruelle et al., 2018). In their observation-based analysis of decadal trends in shelf pCO2, Laruelle et al. (2018) found that coastal waters lag compared to the rise in atmospheric CO2 in most regions. For North American coastal waters, they found that the MAB has an increase in ΔpCO2 of 1.9 ± 3.1 microatmospheres (μatm) per year, a finding which means that in this region surface ocean pCO2 does not increase or else increases at a rate that is substantially slower than in the atmosphere. For the shelves of the Labrador Sea, the Vancouver Shelf, and the SAB, they found rates of 0.68 ± 0.61 μatm per year, 0.83 ± 1.7 μatm per year, and 0.51 ± 0.74 μatm per year, respectively, implying that surface ocean pCO2 does not increase or increases at a slower rate than atmospheric CO2. The only North American coastal region that exhibits a negative trend is the Bering Sea, with –1.1 ± 0.74 μatm per year, meaning that surface ocean pCO2 increases at a faster rate than in the atmosphere. Laruelle et al. (2018) concluded that the lag in coastal ocean pCO2 increase compared to that in the atmosphere in most regions indicates an enhancement in the coastal uptake and export of atmospheric CO2, although they did not investigate alternative explanations.
Trends in coastal ocean uptake of pCO2 are highly variable regionally and result from a complex interplay of factors. In coastal upwelling systems, surface warming will increase the horizontal gradient between cold, freshly upwelled source waters and warm, offshore surface water, leading to a greater tendency for the subduction of upwelled water at offshore surface temperature fronts during periods of persistent and strong upwelling-favorable winds. The cumulative effect of these processes for the North American Pacific Coast may be greater and more persistent CO2 outgassing nearshore and lower productivity offshore as upwelled nitrate is exported before it can be used by the phytoplankton community (Evans et al., 2015a). Rates of warming clearly are faster in higher latitudes, but predicting the net effect of these warming-induced changes in the North American Arctic is not easy. Furthermore, warming in the Arctic leads to reductions in ice cover and longer ice-free periods, both of which directly affect air-sea gas exchange (Bates and Mathis 2009). Another profound effect of Arctic warming is the melting of permafrost, which leads to the release of large quantities of CH4 to the atmosphere, from both the land surface and the coastal ocean (Crabeck et al., 2014; Parmentier et al., 2013).
Changes in wind stress also directly affect air-sea gas fluxes because stronger winds intensify gas exchange. For example, for the North American Atlantic Coast, changes in wind stress were shown to significantly modify air-sea fluxes (Cahill et al., 2016; Previdi et al., 2009). Large-scale changes in wind patterns also affect ocean circulation with a range of implications (Bakun 1990). Upwelling-favorable winds along the North American Pacific Coast have intensified in recent years, especially in the northern parts of the upwelling regimes (García-Reyes et al., 2015; Rykaczewski and Checkley 2008; Rykaczewski et al., 2015; Sydeman et al., 2014), a change which has led to 1) shoaling of subsurface nutrient-rich waters (Aksnesa and Ohman 2009; Bograd et al., 2015), 2) increased productivity (Chavez et al., 2011, 2017; Jacox et al., 2015; Kahru et al., 2015), 3) higher DIC delivery to the surface (Turi et al., 2016), and 4) declining oxygen levels (Crawford and Peña 2016; Peterson et al., 2013; Bograd et al., 2015). In the North American Arctic, late-season air-sea CO2 fluxes may become increasingly more directed toward the atmosphere as Arctic low-pressure systems with storm-force winds occur more often over open water, thus ventilating CO2 respired from the high organic carbon loading of the shallow shelf (Evans et al., 2015b; Hauri et al., 2013; Steiner et al., 2013) and affecting net annual exchanges. The intense warming observed across the North American Arctic also influences mid-latitude weather patterns (Kim et al., 2014), with probable cascading effects on CO2 exchanges through adjustments in the wind field.
16.4.2 Acidification Trends in North America’s Coastal Ocean
Increasing atmospheric CO2 emissions lead to rising atmospheric CO2 levels (see Figure 16.3) and a net ocean uptake of CO2. Since about 1750, the ocean has absorbed 27% of anthropogenic CO2 emissions to the atmosphere from fossil fuel burning, cement production, and land-use changes (Canadell et al., 2007; Le Quéré et al., 2015; Sabine and Tanhua 2010). As a result of this uptake, the surface ocean pCO2 has increased (see Figure 16.3) and oceanic pH, carbonate ion concentration, and carbonate saturation state have decreased (Caldeira and Wickett 2003; Feely et al., 2004, 2009; Orr et al., 2005). Commonly called ocean acidification, this suite of chemical changes is defined more precisely as “any reduction in the pH of the ocean over an extended period, typically decades or longer, that is caused primarily by uptake of CO2 from the atmosphere but also can be caused by other chemical additions or subtractions from the ocean” (IPCC 2011, p. 37). In addition to uptake of CO2 from the atmosphere, variations in DIC concentrations and thus pH can be caused by biological production and respiration. Ocean acidification can significantly affect growth, metabolism, and life cycles of marine organisms (Fabry et al., 2008; Gattuso and Hansson 2011; Somero et al., 2016) and most directly affects marine calcifiers, organisms that precipitate CaCO3 to form internal or external body structures. When the carbonate saturation state decreases below the equilibrium point for carbonate precipitation or dissolution, conditions are said to be corrosive, or damaging, to marine calcifiers. These conditions make it more difficult for calcifying organisms to form shells or skeletons, perform metabolic functions, and survive.
Figure 16.3: Trends in Measured Atmospheric Carbon Dioxide (CO2) and Surface Ocean Partial Pressure of CO22 (pCO2)
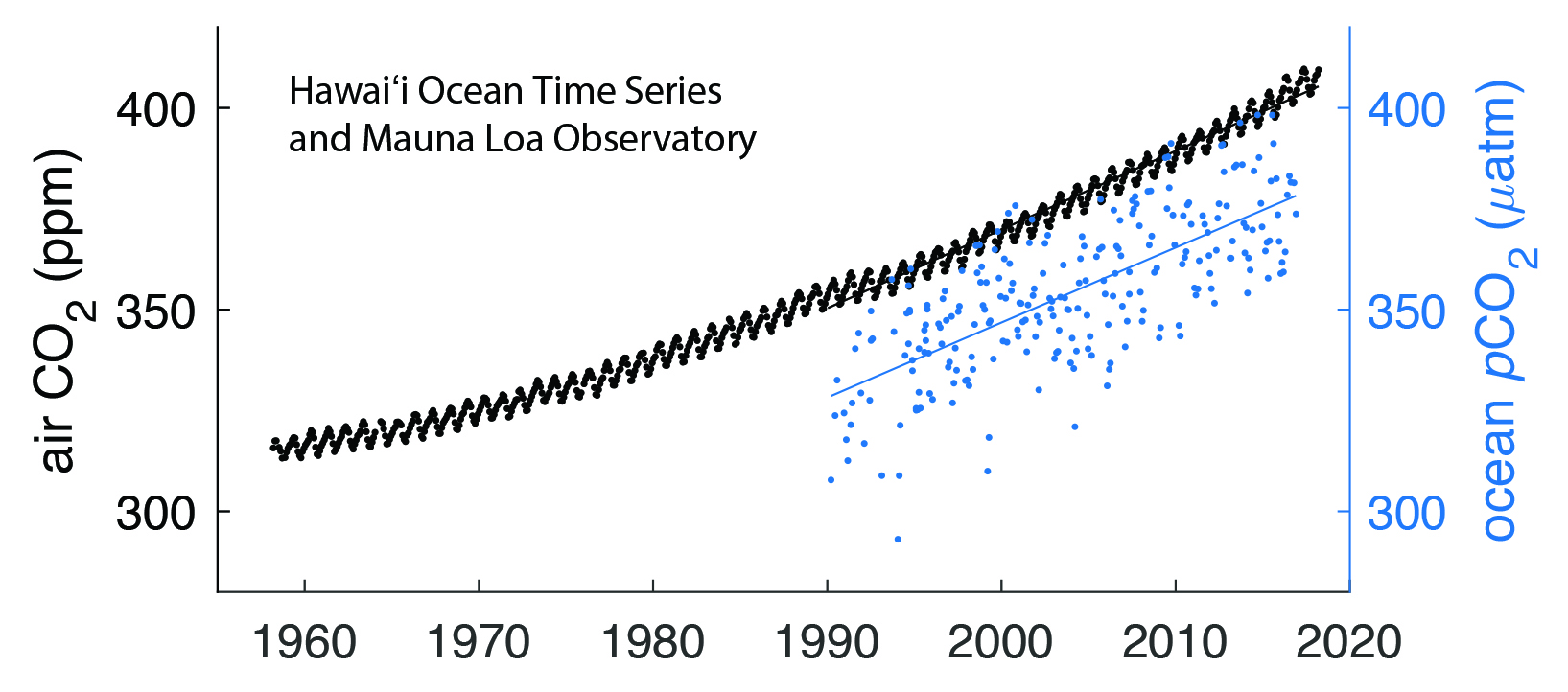
Acidification trends in open-ocean surface waters tend to occur at a rate that is commensurate with the rate of the increase in atmospheric CO2 (see, for example, trends of atmospheric CO2 in comparison to surface ocean pCO2 at the Hawai‘i Ocean Time-series in Figure 16.3). Acidification in coastal waters is more variable because of a combination of changes in circulation and upwelling, larger-amplitude seasonal signals in production and respiration than in the open ocean, and atmospheric CO2 uptake (see Figure 16.4; Feely et al., 2008, 2016, 2018; Chavez et al., 2017). In many coastal regions, pCO2 rises more slowly than in the open ocean (see Section 16.4.1; Laruelle et al., 2018). Along the North American Pacific Coast, climate-driven changes in upwelling circulation result in coastal acidification events. As mentioned in Section 16.4.1, upwelling-favorable winds along this coast have intensified over recent years, especially in the northern parts of the upwelling regimes (García-Reyes et al., 2015; McClatchie et al., 2016; Rykaczewski and Checkley 2008; Rykaczewski et al., 2015; Sydeman et al., 2014). Intensified upwelling supplies deep water to the shelf that is rich in DIC and nutrients but poor in oxygen. Ocean acidification and hypoxia thus are strongly linked ecosystem stressors because low-oxygen, high-CO2 conditions derive from the microbial respiration of organic matter (Chan et al., 2016; Feely et al., 2008, 2016, 2018). In the northern California Current System, pCO2, pH, and aragonite saturation reach levels known to be harmful to ecologically and economically important species during the summer upwelling season (see Ch. 17: Biogeochemical Effects of Rising Atmospheric Carbon Dioxide; Barton et al., 2012, 2015; Bednaršek et al., 2014, 2016, 2017; Feely et al., 2008, 2016; Harris et al., 2013). In the Gulf of Alaska, aragonite saturation drops to near saturation values during the winter months when deep mixing occurs and surface ocean pCO2 exceeds atmospheric pCO2 (Evans and Mathis 2013). Along the Pacific Coast, 50% of shelf waters are projected to experience year-long undersaturation by 2050 (Gruber et al., 2012; Hauri et al., 2013; Turi et al., 2016).
Figure 16.4: Partial Pressure of Carbon Dioxide (pCO2) Data for the Surface Ocean (black) and Overlying Atmosphere (blue) at Five Coastal Sites.
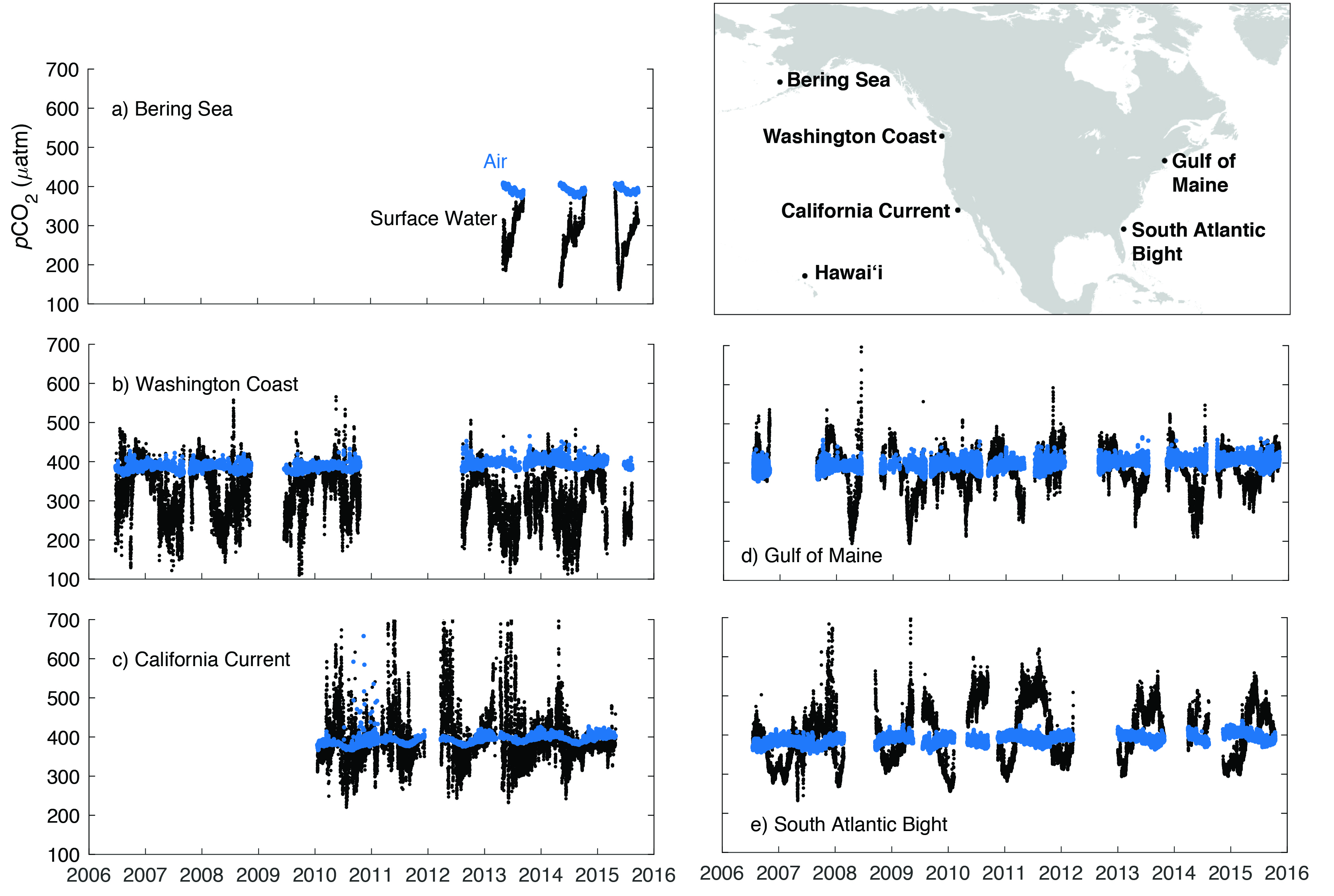
Polar regions are naturally prone to acidification because of their low temperatures (Orr et al., 2005; Steinacher et al., 2009). In many Arctic coastal regions, pH and carbonate saturation state are naturally low relative to lower-latitude coastal settings. These low levels result from higher CO2 solubility, the influence of multiple sources of freshwater (e.g., riverine, glacial melt, and sea ice melt) with varying CO2 chemistries, and the high respiratory DIC content in bottom waters. The Beaufort and Chukchi Sea continental shelves experience inflows of naturally corrosive Pacific seawater with pH as low as 7.6 (Mathis et al., 2011). The main contributing factor to the relatively high rates of acidification in polar waters is retreating sea ice, which adds meltwater from multiyear ice and increases the surface area of open water, thereby enhancing the uptake of atmospheric CO2 (Cai et al., 2010b; Steiner et al., 2013). These factors, in combination with increasing atmospheric CO2 levels, have set a faster pace of ocean acidification in the Arctic than projected trends in other coastal regions (Feely et al., 2009; Mathis et al., 2015a). Models predict annual average aragonite undersaturation (i.e., favoring dissolution) for the Bering Sea and the Chukchi Sea by 2070 and 2030, respectively (Mathis et al., 2015a). The Beaufort Sea upper halocline and deep waters now regularly show aragonite undersaturation (Mathis et al., 2015a; Miller et al., 2014). These chemical seawater signatures are propagated via M’Clure Strait and Amundsen Gulf into the CAA and beyond (Azetsu-Scott et al., 2010; Turk et al., 2016; Yamamoto-Kawai et al., 2013). Model projections based on the IPCC high-CO2 emissions scenario, Representative Concentration Pathway 8.5 (RCP8.5), suggest the Beaufort Sea surface water will become undersaturated with respect to aragonite around 2025 (Steinacher et al., 2009; Steiner et al., 2014). As these conditions intensify, negative impacts on calcifying marine organisms are expected to become a critical issue, reshaping ecosystems and fisheries across the North American Arctic domain (Mathis et al., 2015b; Moore and Stabeno 2015).
In the northern GMx, surface aragonite saturation states typically range from 3.6 to 4.5 and are thus well above the dissolution threshold (Wang et al., 2013; Wanninkhof et al., 2015). Here excessive nutrient inputs from the Mississippi River result in hypoxia and eutrophication-induced acidification of near-bottom waters (Cai et al., 2011; Laurent et al., 2017). Similar to the California Current System, low-oxygen and high-CO2 conditions coincide and derive from microbial respiration of organic matter (Cai et al., 2011; Laurent et al., 2017; Feely et al., 2018). Currently, aragonite saturation states are around 2 in hypoxic bottom waters and thus well above the saturation threshold. Projections suggest that aragonite saturation states of these near-bottom waters will drop below the saturation threshold near the end of this century (Cai et al., 2011; Laurent et al., 2018).
Recent studies indicate that the northern regions of the North American Atlantic Coast (the MAB and GOM) are more prone to acidification than the SAB (Wang et al., 2013; Wanninkhof et al., 2015). Coastal waters in this region have, on average, lower pH and lower aragonite saturation states than more southern coastal regions. These properties are driven primarily by a decrease in mean total alkalinity of shelf water from the SAB northward to the GOM. Seasonal undersaturation of aragonite in subsurface water is occurring in the GOM with photosynthesis and respiration playing a major role in controlling the seasonal variability of aragonite saturation states; dissolution of aragonite might already occur in fall and winter (Wang et al., 2017). With a significant shellfish industry, the GOM displays the lowest pH and aragonite saturation levels along the East Coast in summer (Wang et al., 2013).
See Full Chapter & References