<b>Cooley</b>, S. R., D. J. P. <b>Moore</b>, S. R. Alin, D. Butman, D. W. Clow, N. H. F. French, R. A. Feely, Z. I. Johnson, G. Keppel-Aleks, S. E. Lohrenz, I. B. Ocko, E. H. Shadwick, A. J. Sutton, C. S. Potter, Y. Takatsuka, A. P. Walker, and R. M. S. Yu, 2018: Chapter 17: Biogeochemical effects of rising atmospheric carbon dioxide. In Second State of the Carbon Cycle Report (SOCCR2): A Sustained Assessment Report [Cavallaro, N., G. Shrestha, R. Birdsey, M. A. Mayes, R. G. Najjar, S. C. Reed, P. Romero-Lankao, and Z. Zhu (eds.)]. U.S. Global Change Research Program, Washington, DC, USA, pp. 690-727, https://doi.org/10.7930/SOCCR2.2018.Ch17.
Biogeochemical Effects of Rising Atmospheric Carbon Dioxide
17.3.1 Ocean Acidification
Increased uptake of CO2 by the ocean from the beginning of the Industrial Revolution has led to decreased seawater pH and a lower calcium carbonate (CaCO3) mineral saturation state (see Ch. 16: Coastal Ocean and Continental Shelves, Section 16.4.2). Average pH values for open-ocean surface water have decreased by approximately 0.11 units from a preindustrial mean value of 8.17, equivalent to an increase of about 28% in hydrogen ion concentration (Feely et al., 2004, 2009; Gattuso et al., 2015; Orr et al., 2005). As a result of ocean acidification, the oceanic average concentration of carbonate ion (CO32–) has declined about 16% from preindustrial values (Bopp et al., 2013; Doney et al., 2009; Gattuso et al., 2015). These changes in carbonate chemistry caused by rising atmospheric CO2 have a variety of effects on aquatic life (e.g., Orr et al., 2005 and Kroeker et al., 2013), which is now an area of active research. Thirty-year ocean time-series datasets (e.g., Bates et al., 2014; Dore et al., 2009) provide direct evidence of this phenomenon worldwide (see Figure 17.3). By the end of this century, surface ocean pH is expected to decline by another 0.1 to 0.4 units, and CO32– concentration is expected to decline by as much as 50% compared to preindustrial conditions (see Figure 17.4).
Figure 17.3: Evidence for Ocean Acidification from Ocean Time-Series Stations
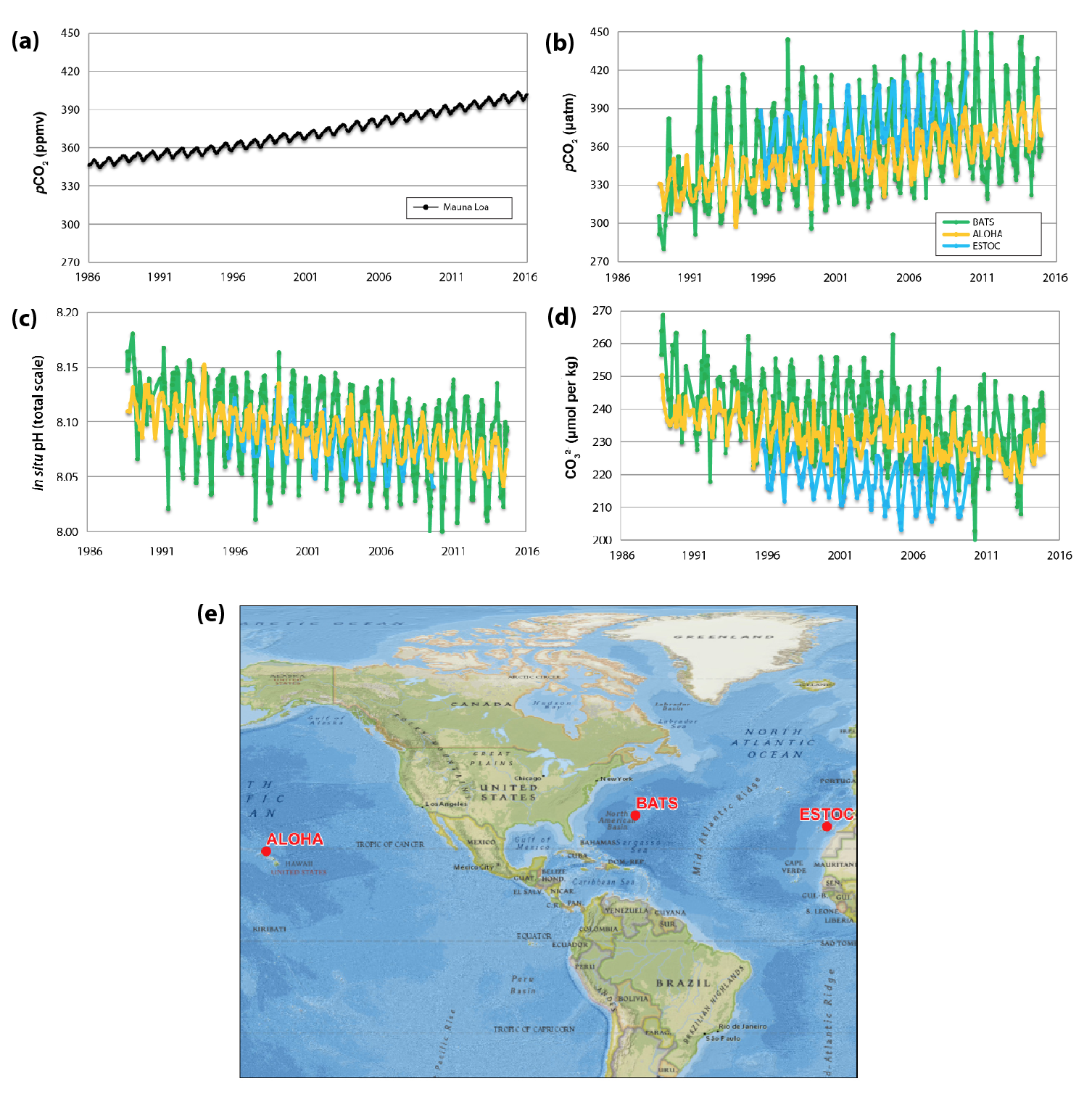
Significant changes in ocean acidity are readily apparent in the subtropical open ocean (see Figure 17.3) and in several coastal locations (Sutton et al., 2016). High-quality, long-term datasets in extremely nearshore locations are limited, but ocean acidification has been documented year-round at time-series observatories near Alaska’s Aleutian Islands and Oahu, Hawai‘i (both open-ocean sites), and the Gulf of Maine and Gray’s Reef off Georgia (both coastal ocean sites; Sutton et al., 2016). Conditions are more variable at coastal and nearshore time-series sites in the California Current and off Washington state (see Ch. 16: Coastal Ocean and Continental Shelves, Section 16.4.2), but they still confirm the presence of significantly acidified conditions during some portions of every year (Sutton et al., 2016). The pH values in coastal waters are much more variable than those in the open ocean (Friedrich et al., 2012; Hofmann et al., 2010; Johnson et al., 2013; Sutton et al., 2016) because of natural processes such as upwelling, biological consumption and release of CO2, temperature- and salinity-driven solubility changes in CO2, or local human inputs of acid-producing substances (see Ch. 16: Coastal Ocean and Continental Shelves, Section 16.4.2). Variable coastal processes make long-term pH trends somewhat harder to discern (Sutton et al., 2016), but these processes can enhance acidification (Doney 2010; Feely et al., 2008; Kelly et al., 2011) far beyond global average projections. The projected long-term average global increase in acidity (decreasing pH values) in the next 20 to 40 years due to atmospheric CO2 (see Figure 17.4) is much greater than the natural variability of pH values observed since monitoring began, underscoring the idea that marine life will face unfamiliar seawater chemistry conditions in the near future.
Figure 17.4: Regional Differences in Acidification Projections

Many coastal margins also suffer from excess anthropogenic nitrogen and phosphorus inputs, which cause algal overgrowth (eutrophication) and, in some cases, increased microbial digestion (remineralization) of organic matter in bottom waters (see Ch. 16: Coastal Ocean and Continental Shelves). These processes further increase CO2 in water, reduce oxygen (i.e., deoxygenation) and pH, and decrease CaCO3 mineral saturation (Cai et al., 2011; Diaz and Rosenberg 2008; Feely et al., 2016; Rabalais et al., 2002). Multiple stresses to coastal zones (e.g., warming, ocean acidification, and deoxygenation) can cause compounding harm to marine ecosystem health (Bijma et al., 2013; Wallace et al., 2014), complicating detection of individual organism impacts and ecosystem trends from acidification (Duarte et al., 2013; Harvey et al., 2013). Future research about how to manage aquatic ecosystems under global change needs to account for the complexity of climate and non-climate drivers and responses in both coastal and ocean environments (Blackford 2010; Riebesell and Gattuso 2015).
17.3.2 Acidification of Freshwater
Inland freshwater can dissolve excess atmospheric CO2 just as seawater does. However, the dearth of long-term, high-precision, high-accuracy carbonate chemistry datasets for even major freshwater bodies like the Laurentian Great Lakes precludes attributing a discernible acidification trend in freshwater bodies to atmospheric CO2 (Phillips et al., 2015). As in coastal waters, local processes also can significantly alter freshwater pH, complicating detection and attribution of changes driven by atmospheric CO2 in lakes and rivers. The effects of acidification-driven changes due to increasing atmospheric CO2 on lake ecosystems have not been determined (Hasler et al., 2015), but species-level studies suggest that, just as in ocean environments, impacts to freshwater organisms could be widespread and yet difficult to forecast (Weiss et al., 2018).
17.3.3 Changes in Ocean Biology and Ocean Biological Processes
Investigations of ocean acidification’s effect on marine life show evidence of a wide range of sensitivities within and across diverse groups of organisms. Calcifying phytoplankton like coccolithophorids as well as multicellular organisms like scleractinian corals, pteropods, foraminifera, bivalves, crustaceans, and gastropods generally show negative but complex responses to ocean acidification, including altered biological processes such as growth, photosynthesis, calcification, and reproductive success (Bednaršek et al., 2016; Hofmann et al., 2010; Kroeker et al., 2013; Riebesell and Tortell 2011; Meyer and Riebesell 2015). Several finfish and shark species display altered risk-taking and hunting behaviors (Hamilton et al., 2014; Munday et al., 2014; Dixson et al., 2014), responses which have been related to changes in olfaction and neurotransmitter levels that result from ocean acidification (Munday et al., 2009; Dixson et al., 2010). Developmental changes in some harvested species such as summer flounder and tuna have also been noted (Chambers et al., 2014; Frommel et al., 2016). Conversely, photosynthesis of phytoplankton (algae), seagrasses, and kelp generally increases (Fu et al., 2007; Hutchins et al., 2013; Riebesell et al., 2007; Mackey et al., 2015), although net responses are highly species-specific and limited by several cellular processes, including species’ carbon capture mechanisms (Mackey et al., 2015). Species responsible for harmful algal blooms are stimulated by changing ocean temperatures, carbonate chemistry, and nutrient ratios, displaying higher growth rates and greater toxin production (Fu et al., 2012). Theory suggests that acidification also may affect bioavailability of nutrients and trace minerals and stoichiometry of biogeochemical processes (Millero et al., 2009), but experimental results are mixed (Breitbarth et al., 2010; Shi et al., 2010). Co-occurrence of elevated temperatures, excessive nutrient inputs, changes in light availability, and increased hypoxia are likely to exacerbate and complicate the effects of ocean acidification on marine organisms or ecosystems (Bijma et al., 2013; Kroeker et al., 2013).
Ocean acidification impacts at the ecosystem level are difficult to predict because of the complexity of species- and population-level responses, but that research is beginning. Population-scale projections of ocean acidification’s effects have been developed for a few high-value, intensively managed single-species fisheries, including Tanner crab (Punt et al., 2016) and sea scallop (Cooley et al., 2015). More broadly, physiological and behavioral changes could alter predator-prey relationships and other species interactions, driving changes in species abundance and composition of ecological communities. Ocean acidification contributes to net loss of corals, and this loss destroys reef habitats and displaces associated marine communities (Hoegh-Guldberg et al., 2007). Ecosystem-scale projections incorporating ocean acidification and other environmental changes are only now being developed for select locations (e.g., California Current, Puget Sound, and northeastern United States; Busch et al., 2013; Fay et al., 2017; Kaplan et al., 2010). Much of the complexity in observed responses lies in 1) different timescales of response relative to the change in ocean acidification, 2) organisms’ abilities to acclimate or genetically adapt, and 3) linkages between ocean acidification and other environmental stressors. Observational (Pespeni et al., 2013; Wootton et al., 2008), integrative (Boyd et al., 2014), and modeling (e.g., Dutkiewicz et al., 2015) studies emphasize the complexity of observed and predicted changes and suggest that future community and functional responses are likely to be more profound than the changes already observed.
17.3.4 Limits in Ocean CO2 Uptake Capacity
Acidification varies with latitude because CO2 solubility depends on temperature, with lower-temperature waters capable of holding more CO2 and thus becoming more readily acidified. Models show that the suite of ocean changes (e.g., circulation, biological productivity, and ventilation) associated with atmospheric CO2 absorption and the thermal effects of CO2 and other greenhouse gases on the ocean are likely to decrease the ocean’s future ability to take up atmospheric CO2 (see Ch. 19: Future of the North American Carbon Cycle, Section 19.6). In the near future, polar ecosystems may change enough to become undersaturated with respect to CaCO3 minerals (Feely et al., 2009; Orr et al., 2005; Steinacher et al., 2010), owing to the large amount of CO2 already dissolved in high-latitude ocean areas. When waters are undersaturated, CaCO3 minerals will not precipitate. Even though low-latitude ocean areas will not become undersaturated with CaCO3 minerals in the future, pH conditions will exceed or have already exceeded the bounds of observed natural variability (see Figure 17.4; Sutton et al., 2016), exposing low-latitude organisms such as warm-swater coral reefs to chemical conditions suboptimal for growth and calcification (Fabricius et al., 2011).
See Full Chapter & References